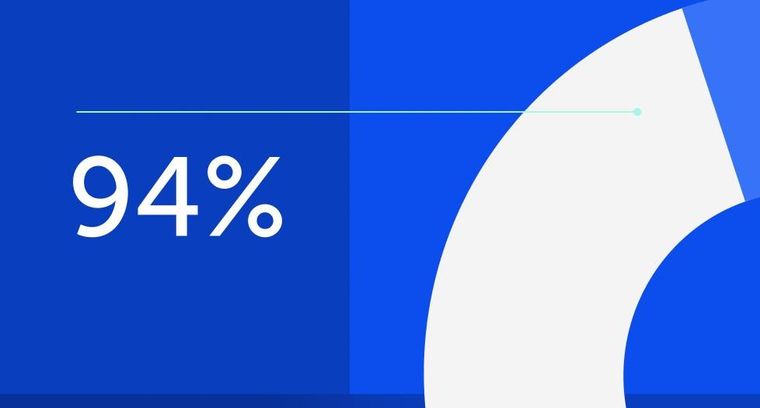
94% of researchers rate our articles as excellent or good
Learn more about the work of our research integrity team to safeguard the quality of each article we publish.
Find out more
REVIEW article
Front. Microbiol., 25 May 2020
Sec. Plant Pathogen Interactions
Volume 11 - 2020 | https://doi.org/10.3389/fmicb.2020.00992
This article is part of the Research TopicDesigning Bio-Formulations Based On Organic Amendments, Beneficial Microbes And Their MetabolitesView all 20 articles
Plant-parasitic-nematodes represent a major threat to the agricultural production of different crops worldwide. Due to the high toxicity of chemical nematicides, it is necessary to develop new control strategies against nematodes. In this respect, filamentous fungi can be an interesting biocontrol alternative. The genus Trichoderma, mycorrhizal and endophytic fungi are the main groups of filamentous fungi studied and used as biological control agents (BCAs) against nematodes as resistance inducers. They are able to reduce the damage caused by plant-parasitic nematodes directly by parasitism, antibiosis, paralysis and by the production of lytic enzymes. But they also minimize harm by space and resource-competition, by providing higher nutrient and water uptake to the plant, or by modifying the root morphology, and/or rhizosphere interactions, that constitutes an advantage for the plant-growth. Besides, filamentous fungi are able to induce resistance against nematodes by activating hormone-mediated (salicylic and jasmonic acid, strigolactones among others) plant-defense mechanisms. Additionally, the alteration of the transport of chemical defense components through the plant or the synthesis of plant secondary metabolites and different enzymes can also contribute to enhancing plant defenses. Therefore, the use of filamentous fungi of the mentioned groups as BCAs is a promising durable biocontrol strategy in agriculture against plant-parasitic nematodes.
Over 4100 species of plant-parasitic nematodes were described to date, among which, a restricted group of genera is considered as major plant-pathogens, whereas others are specific to a more limited range of crops, both causing a high impact to economically important crops. Damage caused by plant nematodes has been estimated as a projected yield loss of 12.3% ($157 billion) worldwide (Singh et al., 2015), more significant damage comparing to invasive insects (around US$70 billion; Bradshaw et al., 2016). The full extent of worldwide nematode damage is likely to be underestimated, since growers are often unaware of their presence because the symptoms caused in the plant are often non-specific, making difficult to attribute crop losses to nematode damage (Jones et al., 2013; Siddique and Grundler, 2018). Additional losses could be related to food quality and visual imperfections associated with infection symptoms (Palomares-Rius et al., 2017).
These nematodes are usually small soil-borne pathogens that can feed on all plant parts (including roots, stems, leaves, flowers and seeds), although most species feed on roots. They need a protrusible stylet for feeding that they use to penetrate the plant cells. The stylet is connected to three to five pharyngeal glands that produce effector molecules, which are often secreted, facilitating penetration, internal migration, and parasitism (Jones et al., 2013; Mejias et al., 2019). Based on their feeding habitats, plant-parasitic nematodes can be broadly categorized as either ectoparasitic or endoparasitic. However, the most important nematodes in terms of crop losses are sedentary endoparasites, the root-knot (Meloidogyne spp.) and cyst nematodes (Heterodera and Globodera spp.; Jones et al., 2013). Nowadays, there is an increasing need to supply more food to the growing population (FAO, 2017); therefore, the control of plant-parasitic nematodes is also a growing global demand.
Given the high economic impact caused by parasitic nematodes, a large number of strategies have been developed for nematode-control in agriculture, including the use of chemical nematicides. However, the need to deploy effective nematode resistance is intensified with the loss of pesticides due to EU regulations (EC No1107/2009) as they are harmful to human health and contaminant for the environment (Zhang et al., 2014, 2017). Strategies associated with biocontrol are proposed as a much safer alternative and highly practicable for plant-parasitic nematodes management. The term biological control (or biocontrol) applies to the use of living organisms to suppress the population density or impact to a specific pest organism, making it less abundant or less damaging than it would otherwise be. Specifically, biological control of nematodes is defined as the regulation of nematode populations and/or a reduction in nematode damage through the action of organisms antagonistic to them, which occur naturally or through the manipulation of the environment or the introduction of antagonists. The organism that suppresses the pathogen is referred to as the biological control agent (BCA) that can interact directly with the pathogen where we include antagonism (antibiosis and competition for nutrients or space among others) or interact indirectly with the pathogen through the host-plant as for example inducing plant-resistance (systemic acquired resistance or SAR and induced systemic resistance or ISR; Pal and Gardener, 2006; Stirling, 2018; Xiang et al., 2018). Nowadays, a wide variety of organisms are known to act as BCAs against plant-parasitic nematodes such as fungi, bacteria, viruses, protists, nematode antagonists and other invertebrates. BCAs, physical methods such as solarization and fallowing or cultural practices as crop rotation were proved in most cases viable applied in combination and/or with reduced doses of chemicals in a scenario of integrated pest management (Khan and Kim, 2007; D’Addabbo et al., 2019). Biocontrol of plant-parasitic nematodes using predatory nematodes dates back to the early 20th century; nevertheless, their potential has only begun to be studied in recent years. Apart from acting as BCAs against plant-parasitic nematodes, these nematodes play a main role in stimulating cycling of plant nutrients, which allow plants to defend themselves more effectively against the attack of pathogens. There are many examples described in the literature, among which we find for example Odontopharynx longicaudata, effective against M. incognita and M. javanica (among others) and M. gaugleri, effective against Heterodera oryzae and M. incognita (among others; Khan and Kim, 2007).
Interestingly, plant growth-promoting rhizobacterias (PGPRs) enhance plant growth by colonizing the plant root system, but some PGPR showed also nematicidal activity against plant-parasitic nematodes. One clear example is the secondary metabolites produced by Pseudomonas fluorescens CHA0 that induce mortality of nematode eggs and second-stage infective juveniles (J2s; Siddiqui and Shaukat, 2003). In a study conducted by Zhao et al. (2018), from 860 strains of bacteria collected from the rhizosphere, 5 showed high efficacy as BCAs against Meloidogyne javanica, i.e., Bacillus cereus, B. subtilis, Pseudomonas putida, P. fluorescens, and Serratia proteamaculans. Hence, PGPRs, in addition to benefit plants growth, have great potential through direct interaction against pathogens such as nematodes.
Plants also have a series of innate defensive strategies to defend themselves from the attack of pests and pathogens in a targeted manner. Once the attack occurred, the plants recognize non-specific molecules of the cell wall of the microorganisms [pathogen- and/or Microbial-Associated Molecular Patterns (PAMPs/MAMPs)], the oral secretions of the herbivores [herbivore-associated molecular patterns (HAMPs)] or signs of cell-plant damage [Damage-Associated Molecular Patterns (DAMPs)], by cell surface pattern recognition receptors (PRRs) (Mithöfer and Boland, 2008; Ramírez-Prado et al., 2018; Hou et al., 2019). Nematode-Associated Molecular Patterns (NAMPs) and its associated receptors have been scarcely described (reviewed in Ali et al., 2018). However, it is generally accepted that during the plant-pathogen interactions, the detection of PAMPs and DAMPs by PRRs triggers a complex network of intracellular signaling cascades leading to defense responses known as PAMP-triggered immunity (PTI). It involves the induction of plant responses coordinated by stress-hormones such as salicylic acid (SA), mostly associated to biotrophic pathogens, and jasmonic acid (JA), and ethylene (ET) against necrotrophic pathogens and herbivores. Additionally, the pathogens generate a diverse repertoire of effector proteins that avoid PTI, a phenomenon known as effector-triggered susceptibility (ETS), against which plants produce specific effector receptors that activate a specific effector-triggered immunity (ETI) (Shigenaga et al., 2017; Ramírez-Prado et al., 2018).
The plant innate immune system comprises local and systemic defense responses. After the attack of a biotroph pathogen and the occurrence of a programmed cell death response in plants, called hypersensitive response (HR), a broad-spectrum immunity to reinfection through the whole plant body, called systemic acquired resistance (SAR) is activated in the plant. SAR signaling is mainly mediated by SA derived compounds, such as methyl-SA (MeSA). Similarly, numerous beneficial microorganisms are capable of inducing in the plant what is known as induced systemic resistance (ISR) against necrotrophic pathogens and herbivores. ISR is regulated mainly by JA/ethylene (ET) signaling, but dependence on SA signaling has also been reported (Van Wees et al., 2008; Martinez-Medina et al., 2016). Systemic resistance mediated by JA is also induced by wounding and is referred to as wound-induced resistance. This type of systemic resistance against the recognition of molecular patterns promotes an improved state of defenses in the plant, which causes the plant to respond much more quickly and effectively against a biotic attack. This process is known as defense priming, sensitization or trained immunity (Reimer-Michalski and Conrath, 2016; Mauch-Mani et al., 2017; Bürger and Chory, 2019).
While fungal pathogens have detrimental effects on plant physiology, mutualistic fungi augment host defense responses to pathogens and herbivores. Fungal partners secrete bioactive molecules such as small peptide effectors, enzymes and secondary metabolites that facilitate colonization and contribute to both symbiotic and defense against pathogenic relationships (Zeilinger et al., 2015). Trichoderma species, arbuscular mycorrhizas, ectomycorrhizas, endophytes, yeasts, and avirulent/hypovirulent strains of certain pathogens are among the main beneficial fungi with biocontrol capacity, also by induction of ISR (Martínez-Medina et al., 2013; Ghorbanpour et al., 2018). In this context, we will focus this review in those studies of the genus Trichoderma, mycorrhizal and endophytic fungi as BCAs for nematode control.
The Trichoderma genus includes a group of anamorphic filamentous fungi whose teleomorphic status has been described in several species, within the Hypocrea genus (Martínez et al., 2015). Trichoderma includes fungi characterized by rapid growth that produce large amounts of conidia whose pigmentation can vary from dark to light green. They grow naturally in different habitats in a wide range of climatic zones from polar to equatorial latitudes; hence, they are the most isolated fungi from the soil (Vinale et al., 2008; Martínez et al., 2015). The species of the genus Trichoderma are ubiquitous colonizers of cellulosic materials and, therefore, can be found wherever there is decaying plant material available, as well as in the plant rhizosphere. However, there are also species specifically limited to some geographic areas (López-Bucio et al., 2015).
A comparative analysis of the genomes of three species of the genus Trichoderma, T. reesei, T. atroviride, and T. virens, has determined that mycoparasitism is the ancestral way of life of this genus and that, subsequently, the rhizosphere colonization evolved. In this respect, the presence of pathogens in the soil and the exudates from the plant-roots favored a shift toward a more generalist way of life (Kubicek et al., 2011, 2019). The comparative genomics of these three specific species suggests that the saprotrophic species T. reesei has a smaller set of genes related to mycoparasitism, thus these genes should have subsequently been lost in T. reesei and other species (Kubicek et al., 2011).
Trichoderma acts as a symbiont capable of colonizing the roots (without reaching the vascular bundle, limited only to the outermost layers) establishing a complex molecular dialogue with the plant-host. This relationship, together with its easy adaptation to various climatic and edaphic conditions, and its growth speed, give this genus an advantage over many other filamentous fungi and make them excellent candidates as BCAs. Currently, several mechanisms of action of Trichoderma are recognized as BCA: mycoparasitism, antibiosis, competition with the pathogen, promotion of plant growth, enhanced plant-tolerance against abiotic stresses and stimulation of its defenses against pathogens (Hermosa et al., 2012; Poveda, 2020).
Mainly used as biofertilizers, mycorrhizal fungi are obligate symbionts of the roots of 97% of the vascular plants. Ectomycorrhizal fungi (ECMF) and arbuscular mycorrhizal fungi (AMF) are the main groups and of great interest in forestry and agronomy, since the AMF are capable of forming symbiotic interactions with 80% of the plant species (Berruti et al., 2016; Ferlian et al., 2018). ECMF mainly include the filum Basidiomycota, some Ascomycota, and very few Zygomycota, capable of colonizing the intercellular space of roots from forest species and forming the so-called Hartig network (Domínguez-Núñez and Albanesi, 2019). Whereas, the AMF, belong to Glomeromycota and establish a root symbiosis intracellularly where they develop specialized structures called arbuscules in intimate contact with the plant cells cytosol (Berruti et al., 2016).
At present, molecular and paleo-biological studies have shown how the origin of AMF and of terrestrial plants occurred simultaneously over time (about 470 million years ago) from epiphytic fungi that grew on the surface of the first vascular plants. They were also necessary for the success of plant terrestrial colonization (Feijen et al., 2018; Rimington et al., 2018; Strullu-Derrien et al., 2018). Thus, ECMF emerged evolutionarily after AMF, once the plants had already colonized the earth (Hoeksema et al., 2018).
Development of a mycorrhizal symbiosis requires continuous signals exchange between the two symbionts, which triggers coordinated differentiation of both partners. Moreover, the intimate interaction of the arbuscules within the root cells requires also a partial suppression of plant defense responses (Liao et al., 2018). Mycorrhizal hyphae are able to colonize places in the soil where plant roots could never reach; besides, hyphae have the ability to absorb nutrients due to active transporters. The fungus can contribute mostly to the supply of phosphorus to the plant, but also other nutrients with low mobility, such as ammonium, potassium, copper, iron, sulfur, molybdenum or zinc. In response, the plant must provide carbohydrates to the fungus, which meet their needs, although it does not have a negative impact on the plant, due to photosynthetic compensation with the fungal supply of nutrients and reduced root development (Berruti et al., 2016; Chen et al., 2018). Moreover, it is widely believed that the inoculation of mycorrhizal fungi provides tolerance to host plants against various stresses like heat, salinity, drought, metals and extremes of temperature (Begum et al., 2019).
The similarities between the fungi structural organs used for nutrient uptake between the biotrophic phytopathogenic fungi (haustoria form) and the AMF (arbuscules form), together with the establishment of a long-term relationship with their host, lead to an incorrect response by the plant. First, the plant recognizes PAMPs/MAMPs type molecular patterns (e.g., chitin), which triggers a generalist defensive response of the PTI or MAMP-triggered immunity type. Subsequently, the recognition of effectors released by the fungus in response to plant defenses activates a more specific defensive response (ETI). This part of the plant responses establishes the main difference between the pathogenic fungus and the symbiont fungi (Jacott et al., 2017). The continuous recognition of the AMF within the colonized cells causes the activation of a JA-mediated SAR through the plant termed as mycorrhizal induced resistance (MIR; Pozo and Azcón-Aguilar, 2007). Due to this mechanism, an increase in the systemic accumulation of different defense compounds, such as phytoalexins, suberin, callose, lignin, ROS, phenolic compounds, terpenoids, PR proteins and sulfur amino acids, was observed in mycorrhizal plants. Accordingly, enhanced enzymatic activity of chitinases, glucanases, proteases, ribonucleases, PALs, chalcone synthases and peroxidases was also detected (Hohmann and Messmer, 2017; Jacott et al., 2017; Hill et al., 2018).
The classic definition of plant endophytes refers to those microorganisms that can be isolated from plant tissues once they have been superficially disinfected. They also do not cause visible damage to plants. This group of microorganisms includes bacterial, archaeal, fungal and protist taxa. Among them, the fungal group play an important role in ecosystems, protecting plants against biotic and abiotic stresses (Lugtenberg et al., 2016; Yan et al., 2019). Numerous studies have indicated that these fungi have a wide biotechnological potential because they biosynthesize molecules, such as enzymes, that can be used as biocontrol and plant-growth-promoting agents as well as in bioremediation, biodegradation or biotransformation (Zheng et al., 2016). In turn, endophytic fungi also have a crucial role in nutrients cycling because, once the plant dies, they behave like saprophytes living from plant tissues (Saikkonen et al., 2015).
Although, endophytic fungi are found in a great variety of plants and ecosystems, there is a strong influence of the local environment in determining endophytic communities; hence, individual endophytes show a different niche occupancy (Glynou et al., 2016), accordingly, they are likely to be affected by future climate change (Giauque and Hawkes, 2016). Despite this geographical distribution, the systemic colonization of plant tissues by endophytic fungi is widely regulated by strong antagonism between the different species (which may run into hundreds), confirming that the systemic effects observed in plants by endophytic fungi are mostly due to chemical movement (Yan et al., 2015).
As mentioned before, endophytic fungi are capable of producing plant-associated metabolites and their analogs, bioactive compounds with diverse biotechnological applications, which have been extensively reviewed by numerous authors (Ludwig-Müller, 2015; Nisa et al., 2015; Saikkonen et al., 2016; Vasundhara et al., 2016; Yan et al., 2018). Among them, compounds with cytotoxic capacity against cancer cells and other diseases, such as taxol, podophyllotoxin, camptothecin, and vinca alkaloids have also been found (Gouda et al., 2016; Uzma et al., 2018). But, they are also capable of producing various mixtures of carbon-based compounds, which are known as volatile organic compounds (VOCs) with a direct application as pesticides (Kaddes et al., 2019).
The use of BCAs from endophytic fungi is a growing area of research as shown from numerous reviews on this topic (Chadha et al., 2015; Card et al., 2016; De Silva et al., 2019; Rabiey et al., 2019). The main mechanisms of direct interaction described include the production of lytic enzymes and/or secondary metabolites (antibiosis) and competition.
Endophytic fungi are also able to induce in plants SAR and ISR against the attack of pests and/or pathogens, but they also need to suppress, at least partially, the defenses of the plants to be able to colonize their tissues (Busby et al., 2016). Epichloë genus includes important endophytic fungi of grasses widely studied for their ability to protect the plants due to the synthesis of different alkaloids. Besides, these fungi enhance plant immunity against chewing insects by promoting endogenous defense responses mediated by the JA pathway (Bastias et al., 2017) and against leaf-fungi as Bipolaris sorokiniana in perennial ryegrass (Lolium perenne) by Epichloë festucae var. lolii (Li et al., 2018). JA-mediated defenses were also observed after the application of the endophytic fungus Chaetomium cochlioides on leaves of Cirsium arvense (Hartley et al., 2015). Furthermore, the application of Penicillium citrinum and Aspergillus terreus in sunflower against the stem rot caused by the fungus Sclerotium rolfsii increased the levels of SA and JA in the plant (Waqas et al., 2015).
Sahebani and Hadavi (2008) reported that under greenhouse conditions, the inoculation of tomato seeds with T. harzianum significantly reduced the level of disease caused by the nematode Meloidogyne javanica; affecting their establishment, development and reproduction (i.e., parameters as the number of galls per plant, the number of egg masses per plant, as well as the number of eggs in each mass). Additionally, they observed a significant reduction in egg hatching, thus demonstrating that this species of the genus Trichoderma has significant potential as BCA against this plant parasite. Similarly, root colonization by T. harzianum impeded nematode performance locally at multiple stages of the parasitism: invasion, galling and reproduction in tomato (Martínez-Medina et al., 2017b).
Moreover, the effect of both the suspension culture and the exudates produced by different species of Trichoderma, in the control of M. incognita on tomato plants has been evaluated. The metabolites produced by the fungus, when growing in liquid culture, have a direct and very effective effect on M. incognita, since it significantly decreased eggs hatching and increased the mortality of the J2. Soil application of culture suspension (containing the fungus spores) affected more negatively to the population of J2s, in addition to increasing plant growth more effectively than fungus exudates (Khan et al., 2018). In both cases, the species with the best results in nematode control was T. harzianum. This study confirms the potential as BCAs against this nematode described previously by Sahebani and Hadavi (2008), but also the potential of other species of this genus as T. viride.
Furthermore, approximately 1 month after the penetration of the J2 from the root-knot nematodes (Meloidogyne spp.), the eggs are laid surrounded by a gelatinous matrix that constitutes an egg-mass secreted by the female, which is considered as a defensive envelope that protects the eggs against microorganisms allowing eggs survival on the soil. According to Sharon et al. (2007), the gelatinous matrix plays a key role in the process of Trichoderma conidial union to the nematode M. javanica, thus explaining the parasitism resulting from some species of this genus of fungi. However, it cannot be generalized for all species since, e.g., the growth of T. harzianum used in this study was inhibited by this matrix, thus not being effective in biocontrol against M. javanica.
The genus Trichoderma has an important potential as BCA, not only against root-knot nematodes but also against cyst-forming nematodes by direct parasitism of both eggs and larvae. It increase the level of extracellular enzymes like chitinase and protease, which allow the penetration of the fungus into the eggs by directly affecting very abundant structural components of the eggshell, thus reducing the number of eggs capable of hatching and therefore, the number of infective J2. Specifically, T. longibrachiatum has a strong inhibitory effect on the hatching of cysts produced by Heterodera avenae, since the spores completely cover the surface of these structures, causing their destruction, which is probably due to the production of enzymes (e.g., chitinases) that caused physiological alterations to the cysts (Zhang et al., 2014). T. longibrachiatum also showed an effect on females and on the development of both eggs and J2s of H. avenae (Zhang et al., 2017), hence T. longibrachiatum can be used as a BCA for the management of H. avenae in selected crop species. Within the cyst-forming nematodes, the specie Globodera pallida has also a high agronomic impact. Contina et al. (2017) used a strain of T. harzianum labeled with GFP as a biomarker to study the fungus-nematode interaction, confirming the reduced infection and reproduction of the nematode. The fungus was capable of negatively affecting both cysts and J2s of G. pallida, however, no effect was observed on the eggs. Besides, T. harzianum established hyphal colonization in the rhizoplane and the rhizosphere of the potato, possibly providing long-term protection to the infection. Therefore, to date, there are numerous studies that demonstrate the ability of the genus Trichoderma to effectively control plant-parasitic nematodes through direct interaction.
Strictly direct mechanisms of mycorrhizal fungi against nematodes are not yet described as they normally act through the plant host, either providing the plant with higher nutrient and water uptake, altering root morphology by increasing root growth and branching, or making the plants more competitive for nutrients and space with other plants, or altered rhizosphere interactions (Schouteden et al., 2015; Wani et al., 2017). Recent studies have confirmed these mechanisms, for example, mycorrhizas (Rhizophagus intraradices and Funneliformis mosseae) reduce tomato root penetration by false root-knot nematode Nacobbus aberrans (Marro et al., 2018), in the same way as the application of Glomus intraradices, G. mosseae, and G. etunicatum against M. javanica in peach trees (Calvet et al., 2001). In contrast, the increment in root colonization by mycorrhizae (Rhizophagus clarus, Claroideoglomus etunicatum, Gigaspora rosea, G. margarita, Scutellospora calospora, and S. heterogama) caused an increase in the population of nematodes Pratylenchus brachyurus in maize crop (Brito et al., 2018) which is opposite to the effect in cotton (Ferreira et al., 2018). All these mechanisms and their effectiveness on the populations and the capacity of infection of the phytoparasitic nematodes will depend closely on the local environmental conditions. In this respect, alterations of potassium, phosphorus and moisture are the main factors negatively involved in the beneficial effect caused by mycorrhizal fungal (Ferreira et al., 2018). Despite some contradictory reports, the use of mycorrhizal fungi for the biological control of plant-parasitic nematodes has been widely studied in numerous crops, as maize (Alvarado-Herrejón et al., 2019), and even in energy crops such as switchgrass (Panicum virgatum) and miscanthus (Miscanthus × giganteus; Emery et al., 2017).
Regarding the nematode-control by endophytic fungi, there is still much speculation on the specific mechanisms by which these fungi antagonize nematodes in most cases, but they are likely quite diverse. Endophytic fungi can directly attack, kill, immobilize, or repel nematodes, confuse them when finding their host, interfere with nurse cell development, compete for resources, or employ a combination of those options (Schouten, 2016). For example, it has been observed how Acremonium implicatum can colonize the xylem of tomato roots and, in the soil, parasitize and destroy the eggs of M. incognita (Yao et al., 2015). Another example is F. oxysporum isolated from banana (Musa spp.) that paralyzes and kills the root-lesion nematode (Pratylenchus goodeyi; Mwaura et al., 2010). They can also produce nematicidal secondary metabolites, as e.g., chaetoglobosin A, chaetoglobosin B, flavipin, 3-methoxyepicoccone and 4,5,6-trihydroxy-7-methylphthalide produced by Chaetomium globosum against M. incognita (Khan et al., 2019), VOCs against M. javanica produced by Daldinia cf. concentrica (Liarzi et al., 2016), or the production of alternariol 9-methyl ether by Alternaria sp. against the pinewood nematode or pine wilt nematode Bursaphelenchus xylophilus (Lou et al., 2016). Only a few reports show opposite results, such as the application of entomopathogenic fungus, e.g., B. bassiana that increased potato nematodes reproduction and potato tubers damage caused by Ditylenchus destructor and D. dipsaci (Mwaura et al., 2017).
Within their capacities as BCAs, it is worth highlighting the ability of filamentous fungi to stimulate plant defenses against pathogens. Calderón et al. (1993) showed for the first time, the induction of ISR mediated by Trichoderma in the grapevine, and one of the first clear demonstrations of induced resistance by Trichoderma showed that the treatment of soil with T. harzianum improved the resistance of bean plants to diseases caused by fungal pathogens Botrytis cinerea and Colletotrichum lindemuthianu (Bigirimana et al., 1997). Subsequently, numerous studies have shown that, by colonizing the plant roots, Trichoderma stimulates their defense mechanisms against numerous phytopathogenic microorganisms, including nematodes (Hermosa et al., 2012). Numerous attempts have been made to control plant-parasitic nematodes with this genus. Trichoderma is capable of inducing resistance in a wide variety of plant species, which leads to transcriptomic, proteomic and metabolomic modifications in the plant (Mukherjee et al., 2012). This systemic defense stimulation prepares the immune response of the plant, allowing a faster response after the priming against the subsequent attack of any kind of pathogen and thus reducing the possibility of disease spread (Hermosa et al., 2012; Bisen et al., 2016; Mendoza-Mendoza et al., 2018). In most cases, this ISR is regulated by a JA/ET signaling, as described by Leonetti et al. (2014). They observed that SA signaling is down-regulated in the early stages of M. javanica infection in tomato roots while, the response mediated by JA/ET is induced in tomato roots treated with the fungus, which indicates that the presence of Trichoderma activates the ISR within the plant. However, it has recently been described that the SA pathway also participates actively in this regulation (Martínez-Medina et al., 2017a, b). Similarly, the induction of defenses in tomato against F. oxysporum f. sp. lycopersici by T. virens is also, mediated through both JA and SA (Jogaiah et al., 2018). Numerous studies have shown how both hormones coordinate the resistance and susceptibility of plants to nematodes (Martínez-Medina et al., 2017a, b). In this respect, the root-knot nematode-plant interaction is highly dynamic, as plant responses differ significantly between the initial stages and the latest infection stages. Yet, the induction of defenses by Trichoderma is a plastic phenomenon. In the first phase, the presence of T. harzianum stimulates faster SA-mediated defense responses, to protect the roots against nematode invasion. In the second phase, when M. incognita suppresses JA-related defenses in the roots, Trichoderma stimulates the expression of JA-dependent defenses, thus antagonizing the suppression of defenses mediated by M. incognita, which leads to a reduction in development and reproduction of the nematodes. Once parasitism is established, the fungus increases the activation of SA-dependent defenses probably through the recognition of eggs, which can contribute to improving defenses against the invasion of new juveniles (Martínez-Medina et al., 2017b). Recently, it was proved not only the ability of T. atroviride to induce systemic resistance in tomato plants against the nematode M. javanica, but its heritability, as the offspring of those plants inoculated with Trichoderma, inherited the resistance against M. javanica (de Medeiros et al., 2017). This heritability extends to the growth promotion induced by this fungus during its interaction with the plant that is accompanied by a significant reduction in the defenses dependent on the SA and JA pathways. In this respect, it is very interesting to highlight how fungal symbiosis and nematode infection induce epigenetic changes in the plants probably guided by small RNAs (Cabrera et al., 2016; de Medeiros et al., 2017; Medina et al., 2017; Ruiz-Ferrer et al., 2018), that includes changes in plants methylomes (Hewezi et al., 2018). It has been recently demonstrated how epigenetic and post-transcriptional modifications control the interactions between the plants and their surrounding microorganisms, that is, these regulatory mechanisms play a key role in promoting plant resistance to pathogens facilitating the establishment of symbiotic relationships. Therefore, it is important to consider the role of these modifications in the adaptation of the plant to environmental stress, including the resistance of the plant to the pathogens and the formation of symbiotic relationships, as well as in the study of the interaction between plant-pathogen-beneficial microorganism, especially because symbiosis and pathogenesis share similar signaling mechanisms (Zogli and Libault, 2017; De Palma et al., 2019). Furthermore, it has been recently described that commercial formulates of some Trichoderma spp. strains induced resistance to M. incognita in tomato in split-root system experiments and additionally, an additive effect to that of the tomato Mi 1.2 resistance gene was also observed (Pocurull et al., 2020). It opens possibilities for integrated pest management of root-knot nematodes with BCAs and other combined control methods.
The high-inoculum dose of Trichoderma can also trigger a SA-mediated SAR response similar to that caused by necrotrophic pathogens (Mukherjee et al., 2012; Bisen et al., 2016); hence, the defense induced by this genus can be mediated by ISR or SAR pathways and also by a complex signaling network that connects the SAR and ISR (Mendoza-Mendoza et al., 2018). Therefore, the induction of the SA, ET and JA pathways in the same plant when colonized by Trichoderma suggest the presence of alternative ISR pathways and a complex signaling network that connects the SAR and ISR defense response pathways. This varies upon the plant species, the Trichoderma strain and the pathogen against which the defense response is directed (Brotman et al., 2012; Nawrocka and Małolepsza, 2013).
The control of mycorrhyzal symbiosis is a finely tuned process that involves multiple regulatory components functioning at different levels. Research over the past few years revealed the critical roles of defense phytohormones in modulating mycorrhizal interactions, from early recognition/colonization events up to the final arbuscular formation and degradation (Liao et al., 2018). The ability of mycorrhizal fungi to activate ISR in the plant against the possible attack of pathogens and/or pests has been reviewed by numerous authors, highlighting Pozo and Azcón-Aguilar (2007), Hohmann and Messmer (2017), and Jacott et al. (2017). Mycorrhizal fungi initially trigger plant defense mechanisms similar to a biotrophic pathogen, but then modulate plant responses for successful colonization. In this sense, SA is known to activate responses against biotrophic pathogens, and it is enhanced by mycorrhizal fungi, although, it is subsequently downregulated, which finally allows the symbiotic interaction. Once the symbiosis is established, the microbe-induced resistance and priming regulated by JA is activated, similarly to the responses controlled by JA and ET pathways against necrotrophic pathogens (Hohmann and Messmer, 2017).
Plant resistance by mycorrhizal fungi against phytopathogenic nematodes have been described, however, in conventional pathogenic-assays, it is difficult to distinguish to what extent the decrease in infection is due solely to systemic resistance or to a direct effect. To try to determine the ability of mycorrhiza induced resistance against nematodes a good strategy is the use of split-root methodology. In this respect, Vos et al. (2012a) showed how the inoculation of tomato roots with F. mosseae in one of the compartments reduced the infection rates of M. incognita and Pratylenchus penetrans in the compartment without fungus, through altered root exudation. The mechanisms through which this increase in the systemic defensive capacity of the roots occurs are related to the activation of genes that encode chitinases, PR proteins, enzymes involved in the detoxification of ROS (whose accumulation occurs during hypertrophy and death cell caused by nematodes) such as glutathione S-transferase or the superoxide dismutase (SOD), enzymes involved in the lignin biosynthesis, and in the shikimate pathway which in turn, produces precursors of various aromatic secondary metabolites against nematodes (Schouteden et al., 2015; Sharma and Sharma, 2017b; Balestrini et al., 2019).
Concerning hormones not directly related to plant defensive responses, it is known that strigolactones, after being exuded from the root, activate hyphal branching and enhanced growth and energy metabolism of symbiotic AMF, and once the symbiosis is established, its production by the plant roots fades (Rochange et al., 2019). Regarding the nematodes, it has been proven that the strigolactones do not contribute to cyst nematode (Heterodera schachtii) hatching but they do play a role in host attraction and subsequent invasion (Martinez et al., 2019). In rice, signaling mediated by strigolactones suppresses jasmonate accumulation and promotes RKN infection (Lahari et al., 2019). In contrast, strigolactones play a positive role in nematode defense in tomato (Xu et al., 2018). Although, further research needs to be undertaken, another way to modulate plant responses by mycorrhization could be altering strigolactones -plants production, which might influence plant-nematode interaction.
Regarding the ECMF, colonization of pines (Pinus thunbergii) promoted a lasting SAR against the pine wilt nematode Bursaphelenchus xylophilus, which is transmitted by beetles of the genus Monochamus and feeds by colonizing the vascular bundles (Nakashima et al., 2016). Interestingly, those fungi do not only act systemically controlling nematodes-infection but enhance plant defenses against pathogens transmitted by the nematodes, as it is the case with the Grapevine Fanleaf Virus (GFLV) transmitted by the nematode vector Xiphinema index, against which AMF R. intraradices induces systemic protection (Hao et al., 2018).
Other examples of systemic resistance produced by AMF are a reduced nematode infection, due to the activity of phenolics and defensive plant enzymes, i.e., peroxidase (PO), polyphenol oxidase (PPO), and SOD, together with a significant reduction of malondialdehyde (MDA) and hydrogen peroxide (H2O2) content in tomato roots inoculated with R. irregularis, that also enhanced plant growth (Sharma and Sharma, 2017a). Similarly, the application of G. mosseae using a split-root system against the sedentary nematode M. incognita and the migratory nematode P. penetrans, in tomato indicated that the infection by both different types of nematodes can be controlled by AMF, but only systemically (Vos et al., 2012b). Similar results were observed in banana (Musa spp.) with split-roots using AMF Glomus intraradices against the migratory nematodes Radopholus similis and Pratylenchus coffeae (Elsen et al., 2008). In other cases, it is not clear whether ISR or a direct mechanism are acting against nematodes or even both mechanisms simultaneously. One example is the reduction of P. penetrans infestation in apple seedlings by AMF (Ceustermans et al., 2018), and of Meloidogyne arenaria in red ginger (Alpinia purpurata) by Gigaspora albida, Claroideoglomus etunicatum, and Acaulospora longula (Da Silva-Campos et al., 2017), or the control of the migratory endoparasitic nematode Scutellonema bradys in yam (Dioscorea spp.) by F. mosseae and Glomus dussii (Tchabi et al., 2016). In contrast, Frew et al. (2018) described that the root application of Claroideoglomus entunicatum, Funneliformis coronatum, R. irregularis, and F. mosseae in wheat produced an increase of the plant-parasitic nematode Pratylenchus neglectus populations. This fact is due to a decrease of root benzoxazinoid glucoside accumulation, an important defense metabolite against the nematode, probably necessary for the root colonization of the fungus itself.
The split root system methodology was also used to study systemic resistance induced by endophytic fungi, as F. oxysporum against the root-knot nematode M. incognita in A. thaliana (Martinuz et al., 2015), in tomato (Dababat and Sikora, 2007), and in banana against the burrowing nematode Radopholus simili (Vu et al., 2006). Another endophytic fungi, Fusarium moniliforme also ISR toward Meloidogyne graminicola in rice (Le et al., 2016). Fusarium spp. achieve this systemic induction due to the synthesis and release of chemical compounds such as 4-hydroxybenzoicacid, indole-3-acetic acid (IAA) and gibepyrone D, which, in addition to being toxic directly to the nematodes, induce defense mechanisms against nematodes in plants (Bogner et al., 2017). But endophytic fungi act also systemically causing the synthesis and transport of chemical defense components in the plant. This is the case of the secondary metabolite forskolin synthesized in the medicinal plant Coleus forskohlii after being inoculated with the endophytic fungi of the stem Phialemoniopsis cornearis and Macrophomina pseudophaseolina and radicular Fusarium redolens, as they enhance the expression of diterpene synthases, that enhances tolerance to M. incognita (Mastan et al., 2019). The ability of P. indica to modify plant stress-hormones has been extensively studied (Le-Xu et al., 2018). Although, several studies show that the application of this fungus against M. incognita (Varkey et al., 2018) and Heterodera glycines (Bajaj et al., 2015) reduces the incidence of the disease and improves plant growth, the exact role of systemic resistance is still unsolved. Similarly, Phialemonium inflatum in cotton against M. incognita (Zhou et al., 2018), Nigrospora sp. in sengon plant (Paraserianthes falcataria) against Meloidogyne spp. (Amin, 2015), Penicillium brefeldianum in melon against M. incognita (Miao et al., 2019) or Fusarium solani and F. oxysporum in tomato against M. incognita (Bogner et al., 2016) were described.
Pochonia chlamydosporia is a nematophagous fungus, but it is also a root endophyte that can colonize the roots of higher plants due to a partial suppression of defensive responses mediated by JA. They also reduce the flowering time, stimulate plant growth and increase seed production in A. thaliana (Zavala-González et al., 2017) and a greater root colonization was directly related to a lower incidence of M. javanica in tomato roots (Escudero et al., 2017). P. chlamydosporia induces systemic resistance against M. javanica in tomato (but not in cucumber) by activating a defensive response mediated by SA (Ghahremani et al., 2019). Interestingly, in the absence of nematodes, it is observed how it promotes systemic resistance mediated by JA in barley (Larriba et al., 2015). Another example in forest plant-species is that the presence of the endophytic fungi Gaeumannomyces cylindrosporus, Paraphoma chrysanthemicola, Phialophora mustea, Exophiala salmonis and Cladosporium cladosporioides modifies the systemic defensive responses reducing the incidence of the nematode Bursaphelenchus xylophilus in pine (Chu et al., 2019). On the other side, the attack of the nematodes can systematically alter the defenses of the plant and prevent its colonization by endophytic fungi. This has been observed in Pinus tabulaeformis after the infection of the pinewood nematode (Bursaphelenchus xylophilus) where the diversity of endophytic fungi in the roots was reduced to about 80% (Chu et al., 2018).
In the same way as for mycorrhizal fungi, strigolactones were necessary for the establishment of the beneficial interaction, at least in the case of the endophytic fungus Mucor sp. on A. thaliana roots. Once the symbiosis is established, the fungi decompose strigolactones in planta and downregulate the expression of strigolactones biosynthesis genes (Rozpądek et al., 2018), preventing, therefore, root invasion by nematodes (Martinez et al., 2019).
Biocontrol strategies for plant-parasitic-nematodes constitute a valid alternative to toxic chemical nematicides. Thereby, a wide diversity of effective strategies based on the use of filamentous fungi used as BCAs are described. They work through two main kinds of mechanisms of action, i.e., those that include the production of secondary metabolites (antibiosis), lytic enzymes, and space competition by Trichoderma. AMF directly acts providing higher nutrient and water uptake to the plant, modifying root morphology and altering the rhizosphere interactions, or competing for photosynthates or colonization/infection sites. Endophytic fungi reduce the attack of the plant-parasitic nematodes by parasitism, by paralyzing the nematodes, through antibiosis, by lytic enzymes production and also by space competition. The second group of action mechanisms are the induction of plant defenses, such as the activation of SAR and ISR by Trichoderma, which seems also heritable. As well as, the modification of roots exudates, strigolactones production, plant secondary metabolites and enzymes production by AMF. Finally, the induction of SAR and ISR, the transport of chemical defense components through the plant and the strigolactones production by endophytic fungi (Figure 1).
Figure 1. Graphical representation of direct and induced resistance against plant-parasitic nematodes by filamentous fungi in a split-root system. The different tissue colonization strategies used by the three fungal groups are schematically represented in the microscopic enlargement of the roots. The mechanisms of fungus to induce resistance in plants either by direct or indirect interaction strategies against nematodes are indicated in colored boxes. The effects of the filamentous fungi on nematodes basically are: To increase J2 mortality, to decrease the hatching and/or nematodes infection rate, as well as to alter nematodes development inside the plant and/or their reproduction. Abbreviations correspond to arbuscular mycorrhizal fungi (AMF), salicylic acid (SA), jasmonic acid (JA), systemic acquired resistance (SAR), induced systemic resistance (ISR), strigolactones (SLs), and mycorrhizal induced resistance (MIR).
The scenario in agricultural systems is very complex as different microorganisms of the rhizosphere and plant-species are present; thus they may respond differently to BCAs. The integration of all microorganisms and plant-responses will determine the final output after the BCA treatment as it is a multifactorial response. Additionally, the strong influence of the local environment that for example, highly determine endophytic communities, is another complexity level to include in the agricultural systems. Therefore, BCAs can have variable control ability when established in different soils, and their success can be difficult to predict. However, there are commercial formulates with some of those filamentous fungi mentioned, effective for nematodes control in experimental conditions for particular crops, but scarce scientific information is still available of experiments on the field. The integration of empirical data knowledge in the field after the treatment with a particular fungi strain or formulate, together with detailed analysis of the plant responses at the molecular level in a particular crop could help to a deep understanding of those complex interactions. Yet, holistic approaches for soil-rhizosphere microbiota detection and characterization of specific plant responses could assist in identifying and predicting major problems as the presence of BCA antagonist in the soil or changes in the rhizosphere biota that may influence the final output. This would allow better practices for an effective nematode-control on a particular crop. Moreover, an in-depth knowledge on the molecular mechanisms of induced resistance by BCAs could allow perhaps to manipulate the plants directly, however, synergic effects derived for example of secondary metabolites induced also by some BCAs should also be integrated. Another question is that usually, BCAs based control methods are slow to implement as they may need time to increase their population up to the level required to be effective for PPN control. Yet, the growers need alternative control methods during this period. The durability of those BCA-based techniques is also a great interrogate because of the mentioned complexity of the agricultural systems.
Perhaps, a feasible scenario would be to design integrated pest management based first on a well stablished method on a ground base, such as those chemically based, and slowly to introduce BCAs together with a detailed monitoring plan with the intention to gradually decrease the dependence on chemical control. We should not also discard the possible implementation of other complementary alternative control methods, including those based on host plant genotypes that may have an enhanced response in combination with BCAs.
In summary, the use of filamentous fungi of the genus Trichoderma, mycorrhizal and endophytic fungi as BCAs is a promising durable biocontrol strategy in agriculture against plant-parasitic nematodes (Figure 1) due mainly to the wide diversity of mechanisms of action described above, that in most of the cases act also in combination. However, there are still major question to address with further research. Moreover, recent reports point to a heritable biocontrol after BCAs infection possibly driven by still not well-known epigenetic mechanisms, opening a new field of research with special applied interest.
JP and PA-U contributed to the search for information and references in different databases, they gathered most of the peer-reviewed manuscript used on this topic, and highly contributed to the manuscript writing. CE contributed to the manuscript writing and the correction and critical reading, as well as to the knowledge on the nematode field.
This work was supported by the Spanish Government (AGL2016-75287-R to CE), by the Castilla–La Mancha Government (SBPLY/17/180501/000287 to CE) and by The Ministry of Science, Innovation and Universities (RED2018-102407-T to CE).
The authors declare that the research was conducted in the absence of any commercial or financial relationships that could be construed as a potential conflict of interest.
Thanks to Darío Rodríguez-Prieto for preparing the infographic-summary of the entire manuscript.
Ali, M. A., Anjam, M. S., Nawaz, M. A., Lam, H.-M., and Chung, G. (2018). Signal Transduction in Plant–Nematode Interactions. Int. J. Mol. Sci. 19:1648. doi: 10.3390/ijms19061648
Alvarado-Herrejón, M., Larsen, J., Gavito, M. E., Jaramillo-López, P. F., Vestberg, M., Martínez-Trujillo, M., et al. (2019). Relation between arbuscular mycorrhizal fungi, root-lesion nematodes and soil characteristics in maize agroecosystems. Appl. Soil Ecol. 135, 1–8. doi: 10.1016/j.apsoil.2018.10.019
Amin, N. (2015). Nematicidal activity of root exudates of sengon plant inoculated with endophytic fungi Nigrospora sp. to control of root-knot nematode Meloidogyne spp. J. Chem. Pharm Res. 7, 307–310.
Bajaj, R., Hu, W., Huang, Y., Chen, S., Prasad, R., Varma, A., et al. (2015). The beneficial root endophyte Piriformospora indica reduces egg density of the soybean cyst nematode. Biol. Control 90, 193–199. doi: 10.1016/j.biocontrol.2015.05.021
Balestrini, R., Rosso, L. C., Veronico, P., Melillo, M. T., De Luca, F., Fanelli, E., et al. (2019). Transcriptomic responses to water deficit and nematode infection in mycorrhizal tomato roots. Front. Microbiol. 10:1807. doi: 10.3389/fmicb.2019.01807
Bastias, D. A., Martínez-Ghersa, M. A., Ballaré, C. L., and Gundel, P. E. (2017). Epichloë fungal endophytes and plant defenses: not just alkaloids. Trends Plant Sci. 22, 939–948. doi: 10.1016/j.tplants.2017.08.005
Begum, N., Qin, C., Ahanger, M. A., Raza, S., Khan, M. I., Ahmed, N., et al. (2019). Role of arbuscular mycorrhizal fungi in plant growth regulation: implications in abiotic stress tolerance. Front. Plant Sci. 10:1068. doi: 10.3389/fpls.2019.01068
Berruti, A., Lumini, E., Balestrini, R., and Bianciotto, V. (2016). Arbuscular mycorrhizal fungi as natural biofertilizers: let’s benefit from past successes. Front. Microbiol. 6:1559. doi: 10.3389/fmicb.2015.01559
Bigirimana, J., De Meyer, G., Poppe, J., Elad, Y., and Höfte, M. (1997). Induction of systemic resistance on bean (Phaseolus vulgaris) by Trichoderma harziamum. Univ. Gent Fac. Landbouwwet. Meded. 62, 1001–1007.
Bisen, K., Keswani, C., Patel, J. S., Sarma, B. K., and Singh, H. B. (2016). “Trichoderma spp.: efficient inducers of systemic resistance in plants,” in Microbial-Mediated Induced Systemic Resistance in Plants, eds D. K. Choudhary and A. Varma (Singapore: Springer), 185–195. doi: 10.1007/978-981-10-0388-2_12
Bogner, C. W., Kamdem, R. S., Sichtermann, G., Matthäus, C., Hölscher, D., Popp, J., et al. (2017). Bioactive secondary metabolites with multiple activities from a fungal endophyte. Microb. Biotechnol. 10, 175–188. doi: 10.1111/1751-7915.12467
Bogner, C. W., Kariuki, G. M., Elashry, A., Sichtermann, G., Buch, A. K., Mishra, B., et al. (2016). Fungal root endophytes of tomato from Kenya and their nematode biocontrol potential. Mycol. Prog. 15:30. doi: 10.1007/s11557-016-1169-9
Bradshaw, C. J., Leroy, B., Bellard, C., Roiz, D., Albert, C., Fournier, A., et al. (2016). Massive yet grossly underestimated global costs of invasive insects. Nat. Commun. 7, 1–8. doi: 10.1038/ncomms12986
Brito, O. D. C., Hernandes, I., Ferreira, J. C. A., Cardoso, M. R., Alberton, O., and Dias-Arieira, C. R. (2018). Association between arbuscular mycorrhizal fungi and Pratylenchus brachyurus in maize crop. Chil. J. Agric. Res. 78, 521–527. doi: 10.4067/S0718-58392018000400521
Brotman, Y., Lisec, J., Méret, M., Chet, I., Willmitzer, L., and Viterbo, A. (2012). Transcript and metabolite analysis of the Trichoderma-induced systemic resistance response to Pseudomonas syringae in Arabidopsis thaliana. Microbiology 158, 139–146. doi: 10.1099/mic.0.052621-0
Bürger, M., and Chory, J. (2019). Stressed out about hormones: how plants orchestrate immunity. Cell Host Microbe 26, 163–172. doi: 10.1016/j.chom.2019.07.006
Busby, P. E., Ridout, M., and Newcombe, G. (2016). Fungal endophytes: modifiers of plant disease. Plant Mol. Biol. 90, 645–655. doi: 10.1007/s11103-015-0412-0
Cabrera, J., Barcala, M., García, A., Rio-Machín, A., Medina, C., Jaubert-Possamai, S., et al. (2016). Differentially expressed small RNAs in Arabidopsis galls formed by Meloidogyne javanica: a functional role for miR390 and its TAS 3-derived tasi RNAs. New Phytol. 209, 1625–1640. doi: 10.1111/nph.13735
Calderón, A. A., Zapata, J. M., Muñoz, R., Pedreño, M. A., and Barceló, A. R. (1993). Resveratrol production as a part of the hypersensitive-like response of grapevine cells to an elicitor from Trichoderma viride. New Phytol. 124, 455–463. doi: 10.1111/j.1469-8137.1993.tb03836.x
Calvet, C., Pinochet, J., Hernández-Dorrego, A., Estaún, V., and Camprubí, A. (2001). Field microplot performance of the peach-almond hybrid GF-677 after inoculation with arbuscular mycorrhizal fungi in a replant soil infested with root-knot nematodes. Mycorrhiza 10, 295–300. doi: 10.1007/PL00009998
Card, S., Johnson, L., Teasdale, S., and Caradus, J. (2016). Deciphering endophyte behaviour: the link between endophyte biology and efficacious biological control agents. FEMS Microbiol. Ecol. 92:fiw114. doi: 10.1093/femsec/fiw114
Ceustermans, A., Van Hemelrijck, W., Van Campenhout, J., and Bylemans, D. (2018). Effect of arbuscular mycorrhizal fungi on Pratylenchus penetrans infestation in apple seedlings under greenhouse conditions. Pathogens 7:76. doi: 10.3390/pathogens7040076
Chadha, N., Mishra, M., Rajpal, K., Bajaj, R., Choudhary, D. K., and Varma, A. (2015). An ecological role of fungal endophytes to ameliorate plants under biotic stress. Arch. Microbiol. 197, 869–881. doi: 10.1007/s00203-015-1130-3
Chen, M., Arato, M., Borghi, L., Nouri, E., and Reinhardt, D. (2018). Beneficial services of arbuscular mycorrhizal fungi - from ecology to application. Front. Plant Sci. 9:1270. doi: 10.3389/fpls.2018.01270
Chu, H., Tang, M., Wang, H., and Wang, C. (2018). Pinewood nematode infection alters root mycoflora of Pinus tabulaeformis Carr. J. Appl. Microbiol. 125, 554–563. doi: 10.1111/jam.13883
Chu, H., Wang, C., Li, Z., Wang, H., Xiao, Y., Chen, J., et al. (2019). The dark septate endophytes and ectomycorrhizal fungi effect on Pinus tabulaeformis Carr. seedling growth and their potential effects to pine wilt disease resistance. Forests 10:140. doi: 10.3390/f10020140
Contina, J. B., Dandurand, L. M., and Knudsen, G. R. (2017). Use of GFP-tagged Trichoderma harzianum as a tool to study the biological control of the potato cyst nematode Globodera pallida. Appl. Soil Ecol. 115, 31–37. doi: 10.1016/j.apsoil.2017.03.010
Da Silva-Campos, M. A., Da Silva, F. S. B., Yano-Melo, A. M., De Melo, N. F., and Maia, L. C. (2017). Application of arbuscular mycorrhizal fungi during the acclimatization of Alpinia purpurata to induce tolerance to Meloidogyne arenaria. Plant Pathol. J. 33, 329–336. doi: 10.5423/PPJ.OA.04.2016.0094
Dababat, A. A., and Sikora, R. A. (2007). Induced resistance by the mutualistic endophyte, Fusarium oxysporum strain 162, toward Meloidogyne incognita on tomato. Biocontrol Sci. Technol. 17, 969–975. doi: 10.1080/09583150701582057
D’Addabbo, T., Laquale, S., Perniola, M., and Candido, V. (2019). Biostimulants for plant growth promotion and sustainable management of phytoparasitic nematodes in vegetable crops. Agronomy 9:616. doi: 10.3390/agronomy9100616
de Medeiros, H. A., de Araújo Filho, J. V., De Freitas, L. G., Castillo, P., Rubio, M. B., Hermosa, R., et al. (2017). Tomato progeny inherit resistance to the nematode Meloidogyne javanica linked to plant growth induced by the biocontrol fungus Trichoderma atroviride. Sci. Rep. 7:40216. doi: 10.1038/srep40216
De Palma, M., Salzano, M., Villano, C., Aversano, R., Lorito, M., Ruocco, M., et al. (2019). Transcriptome reprogramming, epigenetic modifications and alternative splicing orchestrate the tomato root response to the beneficial fungus Trichoderma harzianum. Hortic. Res. 6:5. doi: 10.1038/s41438-018-0079-1
De Silva, N. I., Brooks, S., Lumyong, S., and Hyde, K. D. (2019). Use of endophytes as biocontrol agents. Fungal Biol. Rev. 33, 133–148. doi: 10.1016/j.fbr.2018.10.001
Domínguez-Núñez, J. A., and Albanesi, A. S. (2019). “Ectomycorrhizal fungi as biofertilizers in forestry,” in Biostimulants in Plant Science, eds A. Beck, S. Uhac, and A. A. De Marco (Rijeka: IntechOpen). doi: 10.5772/intechopen.88585
Elsen, A., Gervacio, D., Swennen, R., and De Waele, D. (2008). AMF-induced biocontrol against plant parasitic nematodes in Musa sp.: a systemic effect. Mycorrhiza 18, 251–256. doi: 10.1007/s00572-008-0173-6
Emery, S. M., Reid, M. L., Bell-Dereske, L., and Gross, K. L. (2017). Soil mycorrhizal and nematode diversity vary in response to bioenergy crop identity and fertilization. GCB Bioenergy 9, 1644–1656. doi: 10.1111/gcbb.12460
Escudero, N., Lopez-Moya, F., Ghahremani, Z., Zavala-Gonzalez, E. A., Alaguero-Cordovilla, A., Ros-Ibañez, C., et al. (2017). Chitosan increases tomato root colonization by Pochonia chlamydosporia and their combination reduces root-knot nematode damage. Front. Plant Sci. 8:1415. doi: 10.3389/fpls.2017.01415
Feijen, F. A., Vos, R. A., Nuytinck, J., and Merckx, V. S. (2018). Evolutionary dynamics of mycorrhizal symbiosis in land plant diversification. Sci. Rep. 8:10698. doi: 10.1038/s41598-018-28920-x
Ferlian, O., Biere, A., Bonfante, P., Buscot, F., Eisenhauer, N., Fernandez, I., et al. (2018). Growing research networks on mycorrhizae for mutual benefits. Trends Plant Sci. 23, 975–984. doi: 10.1016/j.tplants.2018.08.008
Ferreira, B. S., Santana, M. V., Macedo, R. S., Silva, J. O., Carneiro, M. A., and Rocha, M. R. (2018). Co-occurrence patterns between plant-parasitic nematodes and arbuscular mycorrhizal fungi are driven by environmental factors. Agric. Ecosyst. Environ. 265, 54–61. doi: 10.1016/j.agee.2018.05.020
Frew, A., Powell, J. R., Glauser, G., Bennett, A. E., and Johnson, S. N. (2018). Mycorrhizal fungi enhance nutrient uptake but disarm defences in plant roots, promoting plant-parasitic nematode populations. Soil Biol. Biochem. 126, 123–132. doi: 10.1016/j.soilbio.2018.08.019
Ghahremani, Z., Escudero, N., Saus, E., Gabaldón, T., and Sorribas, F. J. (2019). Pochonia chlamydosporia induces plant-dependent systemic resistance to Meloidogyne incognita. Front. Plant Sci. 10:945. doi: 10.3389/fpls.2019.00945
Ghorbanpour, M., Omidvari, M., Abbaszadeh-Dahaji, P., Omidvar, R., and Kariman, K. (2018). Mechanisms underlying the protective effects of beneficial fungi against plant diseases. Biol. Control 117, 147–157. doi: 10.1016/j.biocontrol.2017.11.006
Giauque, H., and Hawkes, C. V. (2016). Historical and current climate drive spatial and temporal patterns in fungal endophyte diversity. Fungal Ecol. 20, 108–114. doi: 10.1016/j.funeco.2015.12.005
Glynou, K., Ali, T., Buch, A. K., Haghi Kia, S., Ploch, S., Xia, X., et al. (2016). The local environment determines the assembly of root endophytic fungi at a continental scale. Environ. Microbiol. 18, 2418–2434. doi: 10.1111/1462-2920.13112
Gouda, S., Das, G., Sen, S. K., Shin, H. S., and Patra, J. K. (2016). Endophytes: a treasure house of bioactive compounds of medicinal importance. Front. Microbiol. 7:1538. doi: 10.3389/fmicb.2016.01538
Hao, Z., van Tuinen, D., Fayolle, L., Chatagnier, O., Li, X., Chen, B., et al. (2018). Arbuscular mycorrhiza affects grapevine fanleaf virus transmission by the nematode vector Xiphinema index. App. Soil Ecol. 129, 107–111. doi: 10.1016/j.apsoil.2018.05.007
Hartley, S. E., Eschen, R., Horwood, J. M., Gange, A. C., and Hill, E. M. (2015). Infection by a foliar endophyte elicits novel arabidopside-based plant defence reactions in its host, Cirsium arvense. New Phytol. 205, 816–827. doi: 10.1111/nph.13067
Hermosa, R., Viterbo, A., Chet, I., and Monte, E. (2012). Plant-beneficial effects of Trichoderma and of its genes. Microbiology 158, 17–25. doi: 10.1099/mic.0.052274-0
Hewezi, T., Pantalone, V., Bennett, M., Stewart, C. N., and Burch-Smith, T. M. (2018). Phytopathogen-induced changes to plant methylomes. Plant Cell Rep. 37, 17–23. doi: 10.1007/s00299-017-2188-y
Hill, E. M., Robinson, L. A., Abdul-Sada, A., Vanbergen, A. J., Hodge, A., and Hartley, S. E. (2018). Arbuscular mycorrhizal fungi and plant chemical defence: effects of colonisation on aboveground and belowground metabolomes. J. Chem. Ecol. 44, 198–208. doi: 10.1007/s10886-017-0921-1
Hoeksema, J. D., Bever, J. D., Chakraborty, S., Chaudhary, V. B., Gardes, M., Gehring, C. A., et al. (2018). Evolutionary history of plant hosts and fungal symbionts predicts the strength of mycorrhizal mutualism. Commun. Biol. 1:116. doi: 10.1038/s42003-018-0120-9
Hohmann, P., and Messmer, M. M. (2017). Breeding for mycorrhizal symbiosis: focus on disease resistance. Euphytica 213:113. doi: 10.1007/s10681-017-1900-x
Hou, S., Liu, Z., and Wu, D. (2019). Damage-associated molecular pattern-triggered immunity in plants. Front. Plant Sci. 10:646. doi: 10.3389/fpls.2019.00646
Jacott, C., Murray, J., and Ridout, C. (2017). Trade-offs in arbuscular mycorrhizal symbiosis: disease resistance, growth responses and perspectives for crop breeding. Agronomy 7:75. doi: 10.3390/agronomy7040075
Jogaiah, S., Abdelrahman, M., Tran, L. S. P., and Ito, S. I. (2018). Different mechanisms of Trichoderma virens-mediated resistance in tomato against Fusarium wilt involve the jasmonic and salicylic acid pathways. Mol. Plant Pathol. 19, 870–882. doi: 10.1111/mpp.12571
Jones, J. T., Haegeman, A., Danchin, E. G., Gaur, H. S., Helder, J., Jones, M. G., et al. (2013). Top 10 plant-parasitic nematodes in molecular plant pathology. Mol. Plant Pathol. 14, 946–961. doi: 10.1111/mpp.12057
Kaddes, A., Fauconnier, M. L., Sassi, K., Nasraoui, B., and Jijakli, M. H. (2019). Endophytic fungal volatile compounds as solution for sustainable agriculture. Molecules 24:1065. doi: 10.3390/molecules24061065
Khan, B., Yan, W., Wei, S., Wang, Z., Zhao, S., Cao, L., et al. (2019). Nematicidal metabolites from endophytic fungus Chaetomium globosum YSC5. FEMS Microbiol. Lett. 366:fnz169. doi: 10.1093/femsle/fnz169
Khan, M. R., Ahmad, I., and Ahamad, F. (2018). Effect of pure culture and culture filtrates of Trichoderma species on root-knot nematode, Meloidogyne incognita infesting tomato. Indian Phytopathol. 71, 265–274. doi: 10.1007/s42360-018-0031-1
Khan, Z., and Kim, Y. H. (2007). A review on the role of predatory soil nematodes in the biological control of plant parasitic nematodes. Appl. Soil Ecol. 35, 370–379. doi: 10.1016/j.apsoil.2006.07.007
Kubicek, C. P., Herrera-Estrella, A., Seidl-Seiboth, V., Martinez, D. A., Druzhinina, I. S., Thon, M., et al. (2011). Comparative genome sequence analysis underscores mycoparasitism as the ancestral life style of Trichoderma. Genome Biol. 12:R40. doi: 10.1186/gb-2011-12-4-r40
Kubicek, C. P., Steindorff, A. S., Chenthamara, K., Manganiello, G., Henrissat, B., Zhang, J., et al. (2019). Evolution and comparative genomics of the most common Trichoderma species. BMC Genomics 20:485. doi: 10.1186/s12864-019-5680-7
Lahari, Z., Ullah, C., Kyndt, T., Gershenzon, J., and Gheysen, G. (2019). Strigolactones enhance root-knot nematode (Meloidogyne graminicola) infection in rice by antagonizing the jasmonate pathway. New Phytol. 224, 454–465. doi: 10.1111/nph.15953
Larriba, E., Jaime, M. D., Nislow, C., Martín-Nieto, J., and Lopez-Llorca, L. V. (2015). Endophytic colonization of barley (Hordeum vulgare) roots by the nematophagous fungus Pochonia chlamydosporia reveals plant growth promotion and a general defense and stress transcriptomic response. J. Plant Res. 128, 665–678. doi: 10.1007/s10265-015-0731-x
Le, H. T. T., Padgham, J. L., Hagemann, M. H., Sikora, R. A., and Schouten, A. (2016). Developmental and behavioural effects of the endophytic Fusarium moniliforme Fe14 towards Meloidogyne graminicola in rice. Ann. Appl. Biol. 169, 134–143.
Leonetti, P., Costanza, A., Zonno, M., Molinari, S., and Altomare, C. (2014). How fungi interact with nematode to activate the plant defence response to tomato plants. Commun. Agric. Appl. Biol. Sci. 79, 357–362.
Le-Xu, C. W., Oelmüller, R., and Zhang, W. (2018). Role of phytohormones in Piriformospora indica-induced growth promotion and stress tolerance in plants: more questions than answers. Front. Microbiol. 9:1646. doi: 10.3389/fmicb.2018.01646
Li, F., Christensen, M. J., Gao, P., Li, Y., and Duan, T. (2018). An arbuscular mycorrhizal fungus and Epichloë festucae var. lolii reduce Bipolaris sorokiniana disease incidence and improve perennial ryegrass growth. Mycorrhiza 28, 159–169. doi: 10.1007/s00572-017-0813-9
Liao, D., Wang, S., Cui, M., Liu, J., Chen, A., and Xu, G. (2018). Phytohormones regulate the development of arbuscular mycorrhizal symbiosis. Int. J. Mol. Sci. 19:3146. doi: 10.3390/ijms19103146
Liarzi, O., Bucki, P., Miyara, S. B., and Ezra, D. (2016). Bioactive volatiles from an endophytic Daldinia cf. concentrica isolate affect the viability of the plant parasitic nematode Meloidogyne javanica. PLoS One 11:e0168437. doi: 10.1371/journal.pone.0168437
López-Bucio, J., Pelagio-Flores, R., and Herrera-Estrella, A. (2015). Trichoderma as biostimulant: exploiting the multilevel properties of a plant beneficial fungus. Sci. Hortic. 196, 109–123. doi: 10.1016/j.scienta.2015.08.043
Lou, J., Yu, R., Wang, X., Mao, Z., Fu, L., Liu, Y., et al. (2016). Alternariol 9-methyl ether from the endophytic fungus Alternaria sp. Samif01 and its bioactivities. Braz. J. Microbiol. 47, 96–101. doi: 10.1016/j.bjm.2015.11.004
Ludwig-Müller, J. (2015). Plants and endophytes: equal partners in secondary metabolite production? Biotechnol. Lett. 37, 1325–1334. doi: 10.1007/s10529-015-1814-4
Lugtenberg, B. J., Caradus, J. R., and Johnson, L. J. (2016). Fungal endophytes for sustainable crop production. FEMS Microbiol. Ecol. 92:fiw194. doi: 10.1093/femsec/fiw194
Marro, N., Caccia, M., Doucet, M. E., Cabello, M., Becerra, A., and Lax, P. (2018). Mycorrhizas reduce tomato root penetration by false root-knot nematode Nacobbus aberrans. Appl. Soil Ecol. 124, 262–265. doi: 10.1016/j.apsoil.2017.11.011
Martínez, B., Infante, D., and Peteira, B. (2015). Taxonomía polifásica y variabilidad en el género Trichoderma. Rev. Prot. Veg. 30, 11–22.
Martinez, C. M. E., Guarneri, N., Overmars, H., van Schaik, C., Bouwmeester, H., Ruyter-Spira, C., et al. (2019). Distinct roles for strigolactones in cyst nematode parasitism of Arabidopsis roots. Eur. J. Plant Pathol. 154, 129–140. doi: 10.1007/s10658-019-01691-5
Martínez-Medina, A., Appels, F. V., and van Wees, S. C. (2017a). Impact of salicylic acid-and jasmonic acid-regulated defences on root colonization by Trichoderma harzianum T-78. Plant Signal. Behav. 12:e1345404. doi: 10.1080/15592324.2017.1345404
Martínez-Medina, A., Fernandez, I., Lok, G. B., Pozo, M. J., Pieterse, C. M., and Van Wees, S. C. (2017b). Shifting from priming of salicylic acid-to jasmonic acid-regulated defences by Trichoderma protects tomato against the root knot nematode Meloidogyne incognita. New Phytol. 213, 1363–1377. doi: 10.1111/nph.14251
Martínez-Medina, A., Fernández, I., Sánchez-Guzmán, M. J., Jung, S. C., Pascual, J. A., and Pozo, M. J. (2013). Deciphering the hormonal signalling network behind the systemic resistance induced by Trichoderma harzianum in tomato. Front. Plant Sci. 4:206. doi: 10.3389/fpls.2013.00206
Martinez-Medina, A., Flors, V., Heil, M., Mauch-Mani, B., Pieterse, C. M., Pozo, M. J., et al. (2016). Recognizing plant defense priming. Trends Plant Sci. 21, 818–822. doi: 10.1016/j.tplants.2016.07.009
Martinuz, A., Zewdu, G., Ludwig, N., Grundler, F., Sikora, R. A., and Schouten, A. (2015). The application of Arabidopsis thaliana in studying tripartite interactions among plants, beneficial fungal endophytes and biotrophic plant-parasitic nematodes. Planta 241, 1015–1025. doi: 10.1007/s00425-014-2237-5
Mastan, A., Bharadwaj, R. K. B., Kushwaha, R. K., and Babu, C. S. V. (2019). Functional fungal endophytes in Coleus forskohlii regulate labdane diterpene biosynthesis for elevated forskolin accumulation in roots. Microb. Ecol. 78, 914–926. doi: 10.1007/s00248-019-01376-w
Medina, C., da Rocha, M., Magliano, M., Ratpopoulo, A., Revel, B., Marteu, N., et al. (2017). Characterization of microRNAs from Arabidopsis galls highlights a role for miR159 in the plant response to the root-knot nematode Meloidogyne incognita. New Phytol. 216, 882–896. doi: 10.1111/nph.14717
Mejias, J., Truong, N. M., Abad, P., Favery, B., and Quentin, M. (2019). Plant proteins and processes targeted by parasitic nematode effectors. Front. Plant Sci. 10:970. doi: 10.3389/fpls.2019.00970
Mauch-Mani, B., Baccelli, I., Luna, E., and Flors, V. (2017). Defense priming: an adaptive part of induced resistance. Annu. Rev. Plant Biol. 68, 485–512. doi: 10.1146/annurev-arplant-042916-041132
Mendoza-Mendoza, A., Zaid, R., Lawry, R., Hermosa, R., Monte, E., Horwitz, B. A., et al. (2018). Molecular dialogues between Trichoderma and roots: role of the fungal secretome. Fungal Biol. Rev. 32, 62–85. doi: 10.1016/j.fbr.2017.12.001
Miao, G. P., Han, J., Zhang, K. G., Wang, S. C., and Wang, C. R. (2019). Protection of melon against Fusarium wilt-root knot nematode complex by endophytic fungi Penicillium brefeldianum HS-1. Symbiosis 77, 83–89. doi: 10.1007/s13199-018-0565-0
Mithöfer, A., and Boland, W. (2008). Recognition of herbivory-associated molecular patterns. Plant Physiol. 146, 825–831. doi: 10.1104/pp.107.113118
Mukherjee, M., Mukherjee, P. K., Horwitz, B. A., Zachow, C., Berg, G., and Zeilinger, S. (2012). Trichoderma–plant–pathogen interactions: advances in genetics of biological control. Indian J. Microbiol. 52, 522–529. doi: 10.1007/s12088-012-0308-5
Mwaura, P., Dubois, T., Losenge, T., Coyne, D., and Kahangi, E. (2010). Effect of endophytic Fusarium oxysporum on paralysis and mortality of Pratylenchus goodeyi. Afr. J. Biotechnol. 9, 1130–1134. doi: 10.5897/AJB09.964
Mwaura, P., Niere, B., and Vidal, S. (2017). Application of an entomopathogenic fungus (Beauveria bassiana) increases potato nematodes reproduction and potato tubers damage caused by Ditylenchus destructor and D. dipsaci. Biol. Control 115, 23–29. doi: 10.1016/j.biocontrol.2017.08.012
Nakashima, H., Eguchi, N., Uesugi, T., Yamashita, N., and Matsuda, Y. (2016). Effect of ectomycorrhizal composition on survival and growth of Pinus thunbergii seedlings varying in resistance to the pine wilt nematode. Trees 30, 475–481. doi: 10.1007/s00468-015-1217-0
Nawrocka, J., and Małolepsza, U. (2013). Diversity in plant systemic resistance induced by Trichoderma. Biol. Control 67, 149–156. doi: 10.1016/j.biocontrol.2013.07.005
Nisa, H., Kamili, A. N., Nawchoo, I. A., Shafi, S., Shameem, N., and Bandh, S. A. (2015). Fungal endophytes as prolific source of phytochemicals and other bioactive natural products: a review. Microb. Pathog. 82, 50–59. doi: 10.1016/j.micpath.2015.04.001
Pal, K. K., and Gardener, B. M. S. (2006). Biological control of plant pathogens. Plant Health Instr. 2, 1–25. doi: 10.1094/PHI-A-2006-1117-02
Palomares-Rius, J. E., Escobar, C., Cabrera, J., Vovlas, A., and Castillo, P. (2017). Anatomical alterations in plant tissues induced by plant-parasitic nematodes. Front. Plant Sci. 8:1987. doi: 10.3389/fpls.2017.01987
Pocurull, M., Fullana-Pons, A. M., Ferro, M., Valero, P., Escudero, N., Saus, E., et al. (2020). Commercial formulates of Trichoderma induce systemic plant resistance to Meloidogyne incognita in tomato and the effect is additive to that of the Mi-1.2 resistance gene. Front. Plant Sci. 1:1. doi: 10.3389/fmicb.2019.03042
Poveda, J. (2020). Trichoderma parareesei favors the tolerance of rapeseed (Brassica napus L.) to salinity and drought due to a chorismate mutase. Agronomy 10:118. doi: 10.3390/agronomy10010118
Pozo, M. J., and Azcón-Aguilar, C. (2007). Unraveling mycorrhiza-induced resistance. Curr. Opin. Plant Biol. 10, 393–398. doi: 10.1016/j.pbi.2007.05.004
Rabiey, M., Hailey, L. E., Roy, S. R., Grenz, K., Al-Zadjali, M. A., Barrett, G. A., et al. (2019). Endophytes vs tree pathogens and pests: can they be used as biological control agents to improve tree health? Eur. J. Plant Pathol. 155, 711–729. doi: 10.1007/s10658-019-01814-y
Ramírez-Prado, J. S., Abulfaraj, A. A., Rayapuram, N., Benhamed, M., and Hirt, H. (2018). Plant immunity: from signaling to epigenetic control of defense. Trends Plant Sci. 23, 833–844. doi: 10.1016/j.tplants.2018.06.004
Reimer-Michalski, E. M., and Conrath, U. (2016). Innate immune memory in plants. Semin. Immunol. 28, 319–327. doi: 10.1016/j.smim.2016.05.006
Rimington, W. R., Pressel, S., Duckett, J. G., Field, K. J., Read, D. J., and Bidartondo, M. I. (2018). Ancient plants with ancient fungi: liverworts associate with early-diverging arbuscular mycorrhizal fungi. Proc. R. Soc. B 285:20181600. doi: 10.1098/rspb.2018.1600
Rochange, S., Goormachtig, S., Lopez-Raez, J. A., and Gutjahr, C. (2019). “The role of strigolactones in plant–microbe interactions,” in Strigolactones-Biology and Applications, eds H. Koltai and C. Prandi (Cham: Springer), 121–142. doi: 10.1007/978-3-030-12153-2_4
Rozpądek, P., Domka, A. M., Nosek, M., Ważny, R., Jȩdrzejczyk, R. J., Wiciarz, M., et al. (2018). The role of strigolactone in the cross-talk between Arabidopsis thaliana and the endophytic fungus Mucor sp. Front. Microbiol. 9:441. doi: 10.3389/fmicb.2018.00441
Ruiz-Ferrer, V., Cabrera, J., Martinez-Argudo, I., Artaza, H., Fenoll, C., and Escobar, C. (2018). Silenced retrotransposons are major rasiRNAs targets in Arabidopsis galls induced by Meloidogyne javanica. Mol. Plant Pathol. 19, 2431–2445. doi: 10.1111/mpp.12720
Sahebani, N., and Hadavi, N. (2008). Biological control of the root-knot nematode Meloidogyne javanica by Trichoderma harzianum. Soil Biol Biochem. 40, 2016–2020. doi: 10.1016/j.soilbio.2008.03.011
Saikkonen, K., Mikola, J., and Helander, M. (2015). Endophytic phyllosphere fungi and nutrient cycling in terrestrial ecosystems. Curr. Sci. 109, 121–126. doi: 10.1093/femsec/fiv095
Saikkonen, K., Young, C. A., Helander, M., and Schardl, C. L. (2016). Endophytic Epichloë species and their grass hosts: from evolution to applications. Plant Mol. Biol. 90, 665–675. doi: 10.1007/s11103-015-0399-6
Schouteden, N., De Waele, D., Panis, B., and Vos, C. M. (2015). Arbuscular mycorrhizal fungi for the biocontrol of plant-parasitic nematodes: a review of the mechanisms involved. Front. Microbiol. 6:1280. doi: 10.3389/fmicb.2015.01280
Schouten, A. (2016). Mechanisms involved in nematode control by endophytic fungi. Annu. Rev. Phytopathol. 54, 121–142. doi: 10.1146/annurev-phyto-080615-100114
Sharma, I. P., and Sharma, A. K. (2017a). Co-inoculation of tomato with an arbuscular mycorrhizal fungus improves plant immunity and reduces root-knot nematode infection. Rhizosphere 4, 25–28. doi: 10.1016/j.rhisph.2017.05.008
Sharma, I. P., and Sharma, A. K. (2017b). Physiological and biochemical changes in tomato cultivar PT-3 with dual inoculation of mycorrhiza and PGPR against root-knot nematode. Symbiosis 71, 175–183. doi: 10.1007/s13199-016-0423-x
Sharon, E., Chet, I., Viterbo, A., Bar-Eyal, M., Nagan, H., Samuels, G. J., et al. (2007). Parasitism of Trichoderma on Meloidogyne javanica and role of the gelatinous matrix. Eur. J. Plant Pathol. 118, 247–258. doi: 10.1007/s10658-007-9140-x
Shigenaga, A. M., Berens, M. L., Tsuda, K., and Argueso, C. T. (2017). Towards engineering of hormonal crosstalk in plant immunity. Curr. Opin. Plant Biol. 38, 164–172. doi: 10.1016/j.pbi.2017.04.021
Siddique, S., and Grundler, F. M. (2018). Parasitic nematodes manipulate plant development to establish feeding sites. Curr. Opin. Microbiol. 46, 102–108. doi: 10.1016/j.mib.2018.09.004
Siddiqui, I. A., and Shaukat, S. S. (2003). Suppression of root-knot disease by Pseudomonas fluorescens CHA0 in tomato: importance of bacterial secondary metabolite, 2, 4-diacetylpholoroglucinol. Soil Biol. Biochem. 35, 1615–1623. doi: 10.1016/j.soilbio.2003.08.006
Singh, S., Singh, B., and Singh, A. P. (2015). Nematodes: a threat to sustainability of agriculture. Procedia Environ. Sci. 29, 215–216. doi: 10.1016/j.proenv.2015.07.270
Stirling, G. R. (2018). “Biological control of plant-parasitic nematodes,” in Diseases of Nematodes, eds G. O. Poinar and H. -B. Jansson (Boca Raton, FL: CRC Press), 103–150. doi: 10.1201/9781351071468
Strullu-Derrien, C., Selosse, M. A., Kenrick, P., and Martin, F. M. (2018). The origin and evolution of mycorrhizal symbioses: from palaeomycology to phylogenomics. New Phytol. 220, 1012–1030. doi: 10.1111/nph.15076
Tchabi, A., Hountondji, C. C., Ogunsola, B., Lawouin, L., Coyne, D. L., Wiemken, A., et al. (2016). Effect of two species of arbuscular mycorrhizal fungi inoculation on development of micro-propagated yam plantlets and suppression of Scutellonema bradys (Tylenchideae). J. Entomol. Nematol. 8, 1–10. doi: 10.5897/JEN2015.0149
Uzma, F., Mohan, C. D., Hashem, A., Konappa, N. M., Rangappa, S., Kamath, P. V., et al. (2018). Endophytic fungi-alternative sources of cytotoxic compounds: a review. Front. Pharmacol. 9:309. doi: 10.3389/fphar.2018.00309
Van Wees, S. C., Van der Ent, S., and Pieterse, C. M. (2008). Plant immune responses triggered by beneficial microbes. Curr. Opin. Plant Biol. 11, 443–448. doi: 10.1016/j.pbi.2008.05.005
Varkey, S., Anith, K. N., Narayana, R., and Aswini, S. (2018). A consortium of rhizobacteria and fungal endophyte suppress the root-knot nematode parasite in tomato. Rhizosphere 5, 38–42. doi: 10.1016/j.rhisph.2017.11.005
Vasundhara, M., Kumar, A., and Reddy, M. S. (2016). Molecular approaches to screen bioactive compounds from endophytic fungi. Front. Microbiol. 7:1774. doi: 10.3389/fmicb.2016.01774
Vinale, F., Sivasithamparam, K., Ghisalberti, E. L., Marra, R., Woo, S. L., and Lorito, M. (2008). Trichoderma–plant–pathogen interactions. Soil Biol. Biochem. 40, 1–10. doi: 10.1016/j.soilbio.2007.07.002
Vos, C., Claerhout, S., Mkandawire, R., Panis, B., De Waele, D., and Elsen, A. (2012a). Arbuscular mycorrhizal fungi reduce root-knot nematode penetration through altered root exudation of their host. Plant Soil 354, 335–345. doi: 10.1007/s11104-011-1070-x
Vos, C., Tesfahun, A. N., Panis, B., De Waele, D., and Elsen, A. (2012b). Arbuscular mycorrhizal fungi induce systemic resistance in tomato against the sedentary nematode Meloidogyne incognita and the migratory nematode Pratylenchus penetrans. Appl. Soil Ecol. 61, 1–6. doi: 10.1016/j.apsoil.2012.04.007
Vu, T., Sikora, R., and Hauschild, R. (2006). Fusarium oxysporum endophytes induced systemic resistance against Radopholus similis on banana. Nematology 8, 847–852. doi: 10.1163/156854106779799259
Wani, K. A., Manzoor, J., Shuab, R., and Lone, R. (2017). “Arbuscular mycorrhizal fungi as biocontrol agents for parasitic nematodes in plants,” in Mycorrhiza-Nutrient Uptake, Biocontrol, Ecorestoration, eds A. Varma, R. Prasad, and N. Tuteja (Cham: Springer), 195–210. doi: 10.1007/978-3-319-68867-1_10
Waqas, M., Khan, A. L., Hamayun, M., Shahzad, R., Kang, S. M., Kim, J. G., et al. (2015). Endophytic fungi promote plant growth and mitigate the adverse effects of stem rot: an example of Penicillium citrinum and Aspergillus terreus. J. Plant Interact. 10, 280–287. doi: 10.1080/17429145.2015.1079743
Xiang, N., Lawrence, K. S., and Donald, P. A. (2018). Biological control potential of plant growth-promoting rhizobacteria suppression of Meloidogyne incognita on cotton and Heterodera glycines on soybean: a review. J. Phytopathol. 166, 449–458. doi: 10.1111/jph.12712
Xu, X., Fang, P., Zhang, H., Chi, C., Song, L., Xia, X., et al. (2018). Strigolactones positively regulate defense against root-knot nematodes in tomato. J. Exp. Bot. 70, 1325–1337. doi: 10.1093/jxb/ery439
Yan, J. F., Broughton, S. J., Yang, S. L., and Gange, A. C. (2015). Do endophytic fungi grow through their hosts systemically? Fungal Ecol. 13, 53–59. doi: 10.1016/j.funeco.2014.07.005
Yan, L., Zhao, H., Zhao, X., Xu, X., Di, Y., Jiang, C., et al. (2018). Production of bioproducts by endophytic fungi: chemical ecology, biotechnological applications, bottlenecks, and solutions. Appl. Microbiol. Biotechnol. 102, 6279–6298. doi: 10.1007/s00253-018-9101-7
Yan, L., Zhu, J., Zhao, X., Shi, J., Jiang, C., and Shao, D. (2019). Beneficial effects of endophytic fungi colonization on plants. Appl. Microbiol. Biotechnol. 103, 3327–3340. doi: 10.1007/s0025
Yao, Y. R., Tian, X. L., Shen, B. M., Mao, Z. C., Chen, G. H., and Xie, B. Y. (2015). Transformation of the endophytic fungus Acremonium implicatum with GFP and evaluation of its biocontrol effect against Meloidogyne incognita. World J. Microbiol. Biotechnol. 31, 549–556. doi: 10.1007/s11274-014-1781-2
Zavala-González, E. A., Rodríguez-Cazorla, E., Escudero, N., Aranda-Martinez, A., Martínez-Laborda, A., Ramírez-Lepe, M., et al. (2017). Arabidopsis thaliana root colonization by the nematophagous fungus Pochonia chlamydosporia is modulated by jasmonate signaling and leads to accelerated flowering and improved yield. New Phytol. 213, 351–364. doi: 10.1111/nph.14106
Zeilinger, S., Gupta, V. K., Dahms, T. E., Silva, R. N., Singh, H. B., Upadhyay, R. S., et al. (2015). Friends or foes? Emerging insights from fungal interactions with plants. FEMS Microbiol. Rev. 40, 182–207. doi: 10.1093/femsre/fuv045
Zhang, S., Gan, Y., Ji, W., Xu, B., Hou, B., and Liu, J. (2017). Mechanisms and characterization of Trichoderma longibrachiatum T6 in suppressing nematodes (Heterodera avenae) in wheat. Front. Plant Sci. 8:1491. doi: 10.3389/fpls.2017.01491
Zhang, S., Gan, Y., Xu, B., and Xue, Y. (2014). The parasitic and lethal effects of Trichoderma longibrachiatum against Heterodera avenae. Biol. Control 72, 1–8. doi: 10.1016/j.biocontrol.2014.01.009
Zhao, D., Zhao, H., Zhao, D., Zhu, X., Wang, Y., Duan, Y., et al. (2018). Isolation and identification of bacteria from rhizosphere soil and their effect on plant growth promotion and root-knot nematode disease. Biol. Control 119, 12–19. doi: 10.1016/j.biocontrol.2018.01.004
Zheng, Y. K., Qiao, X. G., Miao, C. P., Liu, K., Chen, Y. W., Xu, L. H., et al. (2016). Diversity, distribution and biotechnological potential of endophytic fungi. Ann. Microbiol. 66, 529–542. doi: 10.1007/s1321
Zhou, W., Wheeler, T. A., Starr, J. L., Valencia, C. U., and Sword, G. A. (2018). A fungal endophyte defensive symbiosis affects plant-nematode interactions in cotton. Plant Soil 422, 251–266. doi: 10.1007/s11104-016-3147-z
Keywords: plant-parasitic-nematodes, Trichoderma, mycorrhizal fungi, endophytic fungi, biocontrol, plant systemic resistance
Citation: Poveda J, Abril-Urias P and Escobar C (2020) Biological Control of Plant-Parasitic Nematodes by Filamentous Fungi Inducers of Resistance: Trichoderma, Mycorrhizal and Endophytic Fungi. Front. Microbiol. 11:992. doi: 10.3389/fmicb.2020.00992
Received: 28 January 2020; Accepted: 23 April 2020;
Published: 25 May 2020.
Edited by:
Santiago Gutierrez, Universidad de León, SpainReviewed by:
Holger Heuer, Julius Kühn-Institut, GermanyCopyright © 2020 Poveda, Abril-Urias and Escobar. This is an open-access article distributed under the terms of the Creative Commons Attribution License (CC BY). The use, distribution or reproduction in other forums is permitted, provided the original author(s) and the copyright owner(s) are credited and that the original publication in this journal is cited, in accordance with accepted academic practice. No use, distribution or reproduction is permitted which does not comply with these terms.
*Correspondence: Carolina Escobar, Y2Fyb2xpbmEuZXNjb2JhckB1Y2xtLmVz
†These authors have contributed equally to this work
Disclaimer: All claims expressed in this article are solely those of the authors and do not necessarily represent those of their affiliated organizations, or those of the publisher, the editors and the reviewers. Any product that may be evaluated in this article or claim that may be made by its manufacturer is not guaranteed or endorsed by the publisher.
Research integrity at Frontiers
Learn more about the work of our research integrity team to safeguard the quality of each article we publish.